31 October 2023: Original Paper
Three-Dimensional Printing of Polycaprolactone/Nano-Hydroxyapatite Composite Scaffolds with a Pore Size of 300/500 μm is Histocompatible and Promotes Osteogenesis Using Rabbit Cortical Bone Marrow Stem Cells
Yang Yang
DOI: 10.12659/AOT.940365
Ann Transplant 2023; 28:e940365
Abstract
BACKGROUND: Many patients have bone defects that exceed the healing size. This study aimed to construct polycaprolactone/nano-hydroxyapatite (PCL/nHA) composite scaffolds with different pore sizes and investigate the osteogenesis and histocompatibility of cortical bone mesenchymal stem cells (BMSCs-C) seeded on it after inoculation.
MATERIAL AND METHODS: After mixing PCL and nHA proportionally, three-dimensional (3D) printing was used to print scaffolds. Porosity, compressive strength, and elastic modulus of PCL/nHA scaffolds were tested. The proliferation of BMSCs-C cells was examined and osteogenesis, chondrogenesis, and adipogenesis were evaluated. BMSCs-C cells were inoculated into 3D printing scaffolds, and histocompatibility between BMSCs-C cells and scaffolds was observed by the cell count kit (CCK-8) assay and LIVE/DEAD staining. After inoculating BMSCs-C cells into scaffolds, alkaline phosphatase (ALP) activity and calcium content were measured.
RESULTS: There was no obvious difference in characteristics between the 3 PCL/nHA composite scaffolds. The porosity, compressive strength, and elastic modulus of the 300/500-μm scaffold were between those of the 300-μm and 500-μm scaffolds. With increasing pore size, the mechanical properties of the scaffold decrease. BMSCs-C cells demonstrated faster growth and better osteogenic, adipogenic, and chondrogenic differentiation; therefore, BMSCs-C cells were selected as seed cells. PCL/nHA composite scaffolds with different pore sizes had no obvious toxicity and demonstrated good biocompatibility. All scaffolds showed higher ALP activity and calcium content.
CONCLUSIONS: The 300/500 μm mixed pore size scaffold took into account the mechanical properties of the 300 μm scaffold and the cell culture area of the 500 μm scaffold, therefore, 300/500 μm scaffold is a better model for the construction of tissue engineering scaffolds.
Keywords: Mesenchymal Stem Cells, Nano-Hydroxyapatite-Collagen, Tissue Engineering, Tissue Scaffolds, Polycaprolactone
Background
Every year, many patients have bone defects that exceed the healing size due to trauma, infection, tumor, and other diseases [1,2]. In this condition, patients usually need surgical intervention. Currently, bone transplantation is still the criterion standard for the treatment of bone defects, but bone transplantation has obvious limitations. First, autologous bone transplantation is limited by the size of the graft, and there is the possibility of infection in the donor area and persistent pain after the operation [3,4]. Second, there is a risk of infectious disease transmission and immune response in allogeneic bone transplantation. However, metal products and bone substitutes also have the risk of stress shielding, wear, and service life, which lead to revision surgery [5]. Bone tissue engineering was developed to solve such problems; seed cells, scaffold materials, and growth factors are 3 essential elements [6].
Bone marrow mesenchymal stem cells (BMSCs) are easy to obtain, have rapid proliferation and low immunogenicity, and can change into osteoblasts, chondrocytes, and adipocytes after induction. Bone marrow mesenchymal stem cells are ideal seed cells; however, there are many hematopoietic stem cells in bone marrow. Therefore, it is difficult to separate mesenchymal stem cells from bone marrow due to contamination of hematopoietic stem cells [7]. Because there are also mesenchymal stem cells in the bone cortex, but there are basically no hematopoietic stem cells, the separation of mesenchymal stem cells from the bone cortex can avoid hematopoietic stem cell contamination [8].
As a carrier of seed cells, scaffold should have good biocompatibility, biodegradability, biological activity, mechanical properties, osteogenic activity, pore structure, and processability [9,10]. Hydroxyapatite (HA) is the main inorganic component of human and animal bones [11]. Synthesized nano-hydroxyapatite (nHA) has been found to have better biological activity and biocompatibility than traditional HA [12]. However, the mechanical strength of nHA is not high, so nHA can be combined with several naturally occurring or artificially synthesized polymers to form composite scaffolds to improve the mechanical properties of nHA [13]. Polycaprolactone (PCL) has biocompatibility and good mechanical strength [14], but its cell adhesion is poor and is used mainly to form composites with other materials to improve biological properties. The microstructure of the scaffold plays an important role in promotion of cell viability and tissue growth. The density and size of the scaffold pores have a significant impact on cell growth and attachment [15]. Research shows that the aperture is close to 300 μm because of its high permeability and vascularization potential, which can promote bone formation [16]. Increasing the diameter of the stent can also improve angiogenesis
Three-dimensional printing (3D printing), also called additive printing or manufacturing, involves the deposition of material on a layer-by-layer basis and then gradually generates a solid model [23]. It applies computer-aided design software that transfers the necessary signals to the 3D printer, which then converts the computerized digital model into a two-dimensional (2D) cross-section, generating solid layers to construct the required objects [23,24]. 3D printing, especially 3D bioprinting, has unique advantages in terms of bracket personalization, accuracy, mechanical strength, pore adjustment, and spatial structure complexity [25]. 3D bioprinting of materials has been applied in biofunctional clinical settings, such as wound repair, drug delivery, tissue engineering, and medical implantation [26,27]. Therefore, 3D bioprinting has been considered a potential novel technology for fabricating complex biological structures in the field of regenerative medicine and tissue engineering [28]. In recent years, a new concept of 4D bioprinting has emerged, which may further advance the field of 3D bioprinting [29,30]. 4D bioprinting has been considered the next-generation additive manufacturing-based fabrication platform used to generate adaptive, intricate, and dynamic hard or soft tissue structure, as well as biomedical devices. [31]. Therefore, 4D printing technology, characterized by responding to external stimuli (pH value, temperature, magnetic field), is becoming increasingly popular and can be considered as the future of 3D printing in the field of tissue engineering [32–34]. However, future clinical transformation still requires interdisciplinary cooperation to address the relevant issues faced in this field.
In summary, the interconnected and multiscale pore structure of the scaffold is more conducive to nutrient transport, metabolic material discharge, new bone growth, and blood vessel growth. In this study, a gradient pore size scaffold containing 80% polycaprolactone (PCL) and 20% nanohydroxyapatite (nHA) was prepared. After the 3D model was made with CAD drawing software, it was printed with the melt deposition printer (FDM printer) to characterize and analyze the support. The rabbit bone marrow mesenchymal stem cells were extracted by adherent culture method and the rabbit bone cortex mesenchymal stem cells were extracted by enzymolysis and loaded on the prepared scaffold to construct the cell–scaffold complex. Finally, the osteogenic performance of the composite scaffold was evaluated by measuring alkaline phosphatase activity, protein concentration, and calcium content.
We established an effective scaffold involving scaffold design, printing, cell culture, and the formation of scaffold cell complexes that demonstrated higher osteogenic ability. This study may provide a theoretical basis for subsequent research.
Material and Methods
PCL/NHA PARTICLE PREPARATION:
A total of 20 g of nHA was added to a triangular cup containing 400 ml of dichloromethane, evenly stirred, and then 20 g of PCL was added and stirred for 1 h. Then, 20 g of PCL was added to the upper solution every 1 h until a total of 80 g of PCL was added. After being fully mixed, the viscous liquid was evenly added to 8 glass Petri dishes, staying in the fume hood for 12 h. After overnight, the culture plate was dried in a drying oven at 70°C for 5 h. Then, the mixing block was removed from the glass culture plate, cut into about 2×2×1 mm cubes, and kept for further analysis.
ESTABLISHMENT OF STL FORMAT 3D MODEL:
First, we set the printing conditions, opened Solidworks, drew the 3D model using the software’s own drawing tool, and exported it to STL format. We opened the Slicer cutting software and set the printing conditions as follows: layer height 0.3 mm, perimeter 3, top of the base layer 3, bottom 3, filling density 20%, and the filling mode was a honeycomb structure. The top/bottom filling model was linear, the wire diameter was 3 mm, the extrusion multiplier was 1, and the extrusion temperature was 200°C.
PREPARATION OF A GRADIENT-APERTURE SCAFFOLD:
After leveling the FDM printer (Regenovo Biotech, Ltd., Hangzhou, China), we connected the printer connector to the laptop and imported the STL format 3D model. We added PCL/nHA particles to the feeding cylinder, used a 400-micron nozzle, and started printing after heating to the set temperature and spinning evenly. After printing the finished product, we continued to replace the STL format 3D model according to the same conditions, printed out the brackets with uniform aperture of 300 μm and 500 μm, respectively, trimmed the scaffold, and then packaged them and sent them for cobalt 60 irradiation sterilization. In this study, the scaffolds were divided into 300-μm scaffold, 500-μm scaffold, and 300/500-μm scaffold.
OBSERVATION OF THE CHARACTERIZATION OF THE SCAFFOLD AND MEASUREMENT OF POROSITY:
After freezing and spraying gold under vacuum, the microscopic morphology of the scaffold was observed and photographed under a scanning electron microscope. The porosity of porous scaffolds was measured by the ethanol substitution method. First, an electronic balance was used to measure the mass of the scaffold (W1). Then, the scaffold was immersed in anhydrous ethanol, the negative pressure was applied in closed state, and the bubbles were seen to overflow. After no more bubbles were generated, the scaffold was removed and filter paper was used to absorb the water on the surface of the scaffold and weighed (W2). The scaffold was added to a 25-ml measuring cylinder, adding anhydrous ethanol to 15 ml with a pipette gun, and the volume of ethanol (V2) was added. The porosity was calculated with the following formula: P=[(W2−W1)/ρ2]/(15−V2)]×100%. In this formula, ρ2 represented the concentration of ethanol (0.79 g/ml).
MECHANICAL PROPERTY TEST OF THE SUPPORT:
Three types of supports were taken, and the appropriate loading module was selected using the universal mechanical property testing machine. The compression rate was set and the compression test was conducted on the material at room temperature. The compression range was about half of the height of the material and the loading rate was 1 mm/min. Five samples were measured for each group. In this study, the elastic modulus was used and calculated as: elastic modulus=stress/strain, where stress=P/A and strain=h/L. In the formula, ‘P’ is the load, ‘A’ is the cross-sectional area of the material, ‘h’ is the compression length, and ‘L’ is the original length of the sample.
BMSCS ISOLATION AND CULTURE:
New Zealand rabbits (age 3–5 days) were purchased from the Guizhou Medical University Animal Experiment Center. In this study, BMSCs were isolated from rabbit bone marrow according to previously reported studies [35,36]. Briefly, bone marrow samples were extracted, prepared, and cultured in Dulbecco’s modified Eagle’s medium (DMEM, Hyclone, Logan, Utah, USA) containing 100 U/ml penicillin/streptomycin and 10% fetal bovine serum (FBS, Gibco, USA). The DMEM medium was changed every 3 days. The bone marrow mesenchymal stem cells (BMSCs) were separated using bone marrow tissue, and bone cortex mesenchymal stem cells (BMSCs-C) were separated using bone tissues, such as ulna and flexor.
IDENTIFICATION OF BONE CORTEX MESENCHYMAL STEM CELLS (BMSCS-C):
Identification with flow cytometry assay was performed, in which isolated cells were passed for 3 generations and digested with 0.25% trypsin, then centrifuged at 1000 r/min for 5 min, resuspended with phosphate buffer solution (PBS) for 5 min, and finally subjected to flow cytometry. Cell surface biomarkers, including CD45, CD29, CD34, and CD44, were detected using the CD45-FITC antibody, the CD29-FITC antibody, the CD34-PE antibody, and the CD44-PE antibody, respectively, according to the manufacturer’s protocols. CellQuest software was used to analyze the positive rate of cell surface biomarkers.
Osteogenic induction, adipogenic induction, and chondrogenesis induction were carried using previously described methods [37]. Osteogenic differentiation was induced by Alizarin red S staining (SolariBio, Beijing, China), adipogenic differentiation was induced by oil red O staining (SolariBio, Beijing, China), and chondrogenesis induction was induced by Alcian blue staining (Cyagen BioSci, Santa Clara, CA, USA).
CYTOCOMPATIBILITY EVALUATION OF THE PCL/NHA SCAFFOLD:
BMSCs-C were cultured in passage 3 and then cultured for cytocompatibility with PCL/nHA scaffold, using cell counting kit 8 (CCK-8) assay and the LIVE/DEAD staining method. BMSCs-C cells from passage 3 were adjusted to the density of 2×106/ml, seeded the PCL/nHA scaffold in a 96-well plate, and then cultured for 1 day, 4 days, and 7 days at 37°C for evaluation of cell proliferation. At the end of each time point, BMSCs-C were incubated with a CCK-8 solution (Sigma, St. Louis, MO, USA) at a concentration of 10% for 2 h. Finally, the optical density (OD) of BMSCs-C was detected at a wavelength of 450 with an ELISA reader (Hiperion MPR4, Germany). For LIVE/DEAD staining, BMSCs-C cells were cultured as in the CCK-8 assay for 28 days and stained with the staining solution (10 mal PBS solution containing 5 μl of AM calcine and 20 μl of ethidium homodimer-1) for 30 min. Finally, the images of LIVE/DEAD stained BMSCs-C cells were captured using laser confocal microscopy (FV1000, Olympus, Tokyo, Japan).
EVALUATION OF ALKALINE PHOSPHATASE (ALP) ACTIVITY AND CALCIUM CONTENT:
BMSCs-C were cultured in passage 3, seeded on a 300-μm scaffold, a 500-μm scaffold, and a 300/500-μm scaffold, with a density of 100 000 cells per scaffold, and cultured for 3 days. After 3 days of culture, BMSCs-C cells were subjected to osteogenic induction for 1 day, 7 days, 14 days, and 21 days. The induced BMSCs-C cells at these time points were then washed with PBS 3 times, digested with 0.25% trypsin, and centrifuged at 1000 r/min for 10 min. The cells obtained were then treated with 0.1% Triton X-100 for 30 min on ice at 4°C, and centrifuged at 5000 r/min for 10 min. Finally, the cell supernatants were analyzed for alkaline phosphatase activity and calcium content.
The activity of alkaline phosphatase (ALP) and calcium content were evaluated according to the methods of previous studies [38,39], with some modifications. ALP activity and calcium content were examined according to the protocols of the alkaline phosphatase assay kit (Cat. No. A059-2-2, Nanjing Jiancheng Bioengineering Ins., Nanjing, China) and calcium assay kit (Cat. No. C004-2-1, Nanjing Jiancheng Bioengineering Ins.). Briefly, BMSCs-C cells were rinsed using PBS and lysed with 0.1% Triton X-100, as previously described, and centrifuged, and the supernatant was removed to obtain the cell pellet. For measurement of ALP activity, the BMSCs-C pellet was dissolved in the reaction solution at 37°C for 15 min. The absorbance of light of the BMSCs-C in plates was measured using a microplate reader (Hiperion MPR4, Germany) at 520 nm for the quantitative measurement of ALP activity. For calcium content measurement, the obtained pellet was lysed with lysis buffer and centrifuged at 10 000 r/min for 15 min to collect the supernatants. The supernatants were treated with the kit’s reaction solution to measure the calcium content. The absorbance of light was measured with a microplate reader (Hiperion MPR4, Germany) at 610 nm to detect the quantitative calcium content. ALP activity and calcium content in BMSCs-C were calculated with reference to an established standard curve.
STATISTICAL ANALYSIS:
Data were expressed as mean±standard deviation (SD) and were analyzed with SPSS 22.0 (IBM, Chicago, USA) statistical software. One-way analysis of variance (ANOVA) followed by Turkey’s post hoc test was used to compare differences between groups (such as different groups of pore sizes). When the data variance was homogeneous, the LSD method was used to compare the data, and when the data did not meet the homogeneity of variance after Levent test, the Dunnett’s T3 test was used to compare the data. A
Results
GENERAL CHARACTERISTICS AND ULTRASTRUCTURE OF PCL/NHA COMPOSITE SCAFFOLDS:
In this study, 3D printing technology was used to model PCL/nHA scaffolds, including 300-μm pore size scaffolds, 500-μm pore size scaffolds, and 300/500-μm mixed pore size scaffolds. A three-dimensional model diagram was established using Solidworks. The 300/500-μm scaffold had a mixed aperture, with 500-μm large pore size and 300-μm small pore size with a 1: 2 proportion, and the internal pores were interconnected (Figure 1A).
With an increase in the concentration of nHA, the printing difficulty of the PCL/nHA composite scaffold also increases, making it easily blocking the material barrel. The nHA content in this stent was 20%, and the printed PCL/nHA stent was white, with a size of 0.6×0.5×0.5 cm. In general, there was no obvious difference between the 3 PCL/nHA composite scaffolds. The fibers were evenly arranged, the texture was tough, and the strength was good (Figure 1B).
Scanning electron microscopy showed that the 3 scaffolds were full of pores, the pores were completely connected, and the fibers were organized in a regular and orderly manner and staggered with each other (Figure 1B). There was no gap on the fiber surface, the fiber space and diameter were relatively uniform, and the pore size was 300–500 μm, which meets the design requirements. The surface of the scaffold fiber was rough, which is conducive to cell adsorption.
POROSITY MEASUREMENT OF PCL/NHA COMPOSITE SCAFFOLDS WITH DIFFERENT PORE SIZES:
The results showed that the porosity of all scaffolds was over 50%. Porosity that is too high will reduce the mechanical strength of the scaffold, but porosity that is too low will reduce the osteogenesis efficiency. When the porosity of the engineering scaffold is over 50%, it is more suitable for osteogenesis [40]. Therefore, these 3 kinds of scaffolds met the engineering scaffold standards, and the porosity increased with increasing scaffold pore size. The scaffold with a pore size of 500 μm had the highest porosity and the scaffold with a pore size of 300 μm had the lowest porosity, while the mixed pore size scaffold was in the middle (Figure 1C).
EVALUATION OF MECHANICAL PROPERTIES OF PCL/NHA COMPOSITE SCAFFOLDS:
As shown in Figure 1C, the compression strengths of the 300-μm scaffold, 300/500-μm scaffold, and 300/500-μm scaffold were 12.89±2.07 MPa, 11.31±1.14 MPa, and 6.8±0.87 MPa, respectively. The statistical results of the one-way ANOVA showed that the compression strength of the 300/500-μm scaffold was significantly lower than that of the 500-μm scaffold (Figure 1C, P<0.01). However, there were no significant differences in compression strength between the 300/500-μm scaffold group and the 300-μm scaffold group (Figure 1C, P>0.05).
The elastic modulus of the 300-μm scaffold, 300/500-μm scaffold, and 300/500-μm scaffold was 50.61±6.45 Mpa, 40.21±8.95 Mpa, and 24.17±6.66 Mpa, respectively (Figure 1C). The one-way ANOVA findings showed that the elastic modulus of the 500-μm scaffold group and 300/500-μm group was significantly lower compared to that of the 300-μm scaffold group (Figure 1C, both P<0.01). The elastic modulus of 300/500-μm scaffold group was markedly higher compared to that of the 500-μm scaffold group (Figure 1C, P<0.05).
The slope of the strain/stress curve of the 500-μm scaffold was lower compared to 300/500-μm scaffold and the 300-μm scaffold (Figure 1D). Therefore, with increasing pore size, the mechanical properties of the scaffold decreased, and a 300/500-μm scaffold was preserved in the 500-μm scaffold structure. Compared to the 300-μm scaffold, the mechanical properties of 300/500-μm scaffold only slightly decreased.
CELL CULTURE AND PASSAGE OF BMSCS:
BMSCs-M can be seen under the 100× microscope, and the cell morphology was mainly spindle, spindle, and polygonal, the degree of cell fusion was more than 90%, and growth was rapid. BMSCs-C cultured in the same batch were easy to observe in primary culture because the medullary cavity was washed, with few blood cells. The morphology of BMSCs-C was consistent with that of BMSCs-M, with a cell fusion of 70%. However, the number of BMSCs-C was less than that of BMSCs-M (Figure 2A). When the degree of cell fusion reached 80%, the BMSCs-M grew rapidly. However, when BMSCs-M were subcultured for generation of P5, the cell morphology was significantly prominent and increased compared to the primary cell cytoplasm, with a slower growth rate and a tendency of aging. The morphology of BMSCs-C cultured in the same batch also had an aging tendency, but the growth rate was higher and the degree of aging was lower than that of BMSCs-M. Therefore, BMSCs-C cells were selected as seed cells to inoculate onto the PCL/nHA composite scaffolds.
IDENTIFICATION OF BMSCS-C CELLS BY FLOW CYTOMETRY:
Cultured BMSCs-C cells in the fifth generation were selected to identify the positive expression rate of surface biomarkers (D29, CD44, CD34, and CD45) on BMSCs-C cells, with flow cytometry. The results showed that BMSCs-C cells expressed high levels of CD29 (98.81%) and CD44 (98.15%), and low levels of CD34 (1.24%) and CD45 (0.77%) (Figure 2B). Therefore, the isolated cells were BMSCs-C cells, not vascular endothelial cells or hematopoietic stem cells.
IDENTIFICATION OF OSTEOGENIC, ADIPOGENIC, AND CHONDROGENIC DIFFERENTIATION:
BMSCs-C proliferation was slowed after osteogenesis induction, and cells changed from a long spindle to a short spindle and a spindle. After 4 weeks of osteogenesis induction, BMSCs-C cells were stained with Alizarin red. Under the light microscope, the whole field of vision was brick-red and mineralized nodules were scattered throughout (Figure 2C). This result suggests that there were large amounts of inorganic calcium deposits in BMSCs-C cells and mineralized nodules after osteogenesis induction.
After lipogenic induction, the BMSCs-C cells were filled with lipid droplets of different sizes, and cell volume increased significantly. Two weeks after adipogenic induction, BMSCs-C cells were stained with oil red O, which showed that fat droplets of different sizes in cells were stained orange with oil red O (Figure 2C).
After chondrogenic induction of BMSCs-C cells, the cells gathered into a spherical shape and the cell clusters gathered more and more closely over time. After 3 weeks of chondrogenic induction, BMSCs-C cells were stained with Alsian blue and the results showed that the intra-acid mucopolysaccharide in cartilage tissue was stained light green (Figure 2C).
THE PCL/NHA COMPOSITE SCAFFOLDS DID NOT HAVE OBVIOUS TOXICITY:
The CCK-8 assay was used to detect cell proliferation on the 1st, 4th, and 7th day after inoculation of BMSCs-C cells on the PCL/nHA composite scaffolds. A one-way analysis of variance (ANOVA) test was used to compare the OD values measured in each group on the same day. The results showed that the cell proliferative viability of BMSCs-C cells in the 300/500-μm scaffold group on the first day after inoculation was obviously lower compared to that of the NC group (Figure 3A, P<0.05). BMSCs-C cells in the 300-μm scaffold group on the first day after inoculation demonstrated greater proliferative viability compared to that in the NC group (Figure 3A, P<0.05). However, there were no significant differences for the proliferative capacity of BMSCs-C cells in any groups (Figure 3A, P>0.05). These results suggest that PCL/nHA composite scaffolds had no obvious toxicity.
THE PCL/NHA COMPOSITE SCAFFOLDS DEMONSTRATED GOOD BIOCOMPATIBILITY:
BMSCs-C cells were inoculated on PCL/nHA scaffolds with different pore sizes to form a PCL/nHA composite scaffold-BMSCs-C cell complex. Then, the BMSCs-C cells were cultured until the 28th day and the LIVE/DEAD staining was performed (green fluorescent cells were living cells and red fluorescent cells were dead cells). Confocal microscope images showed that a large number of BMSCs-C cells grew on the scaffolds of all these scaffolds with different pore sizes (Figure 3B). Cells adhered to the surface of the scaffolds to grow, and the number of living cells was significantly higher than the number of dead cells (Figure 3B). The above results suggest that the scaffold had good biocompatibility.
THE PCL/NHA COMPOSITE SCAFFOLDS PROMOTED OSTEOGENESIS:
At the late stage of osteogenesis, the secretion of ALP increased, which is a marker of early osteogenesis differentiation. The activity of ALP was lower on the first day of each group. One-way ANOVA showed that after 7 and 14 days of osteogenesis induction, the ALP activity (Figure 4A) and calcium content (Figure 4B) of each scaffold group were significantly higher than that of the scaffold-free NC group (P<0.05). The activity of ALP (Figure 4A) and the calcium content (Figure 4B) of the 300/500-μm pore size scaffold and the 300-μm pore size scaffold were significantly higher than that of the 500-μm pore size scaffold (P<0.05). However, there was no significant difference for ALP activity and calcium content between the 300/500-μm pore size scaffold and 300-μm pore size scaffold (Figure 4A, 4B, P>0.05). Therefore, the 300/500-μm pore size scaffold and the 300-μm pore size scaffold both had a small pore size, indicating that small pore sizes are conducive to calcium accumulation and early osteogenesis. The above results show that PCL/nHA composite scaffolds have a strong ability to promote osteogenesis.
Discussion
In this study, BMSCs-C cells were selected and inoculated into the PCL/nHA composite scaffold produced by 3D printing technology to construct the BMSCs-C-PCL/nHA composite scaffold and verified the effect of the pore size structure on the mechanical properties of the scaffolds and on cell proliferation and osteogenesis.
The printed scaffold was made of a mixture of nHA and PCL, and the composition was not complex. PCL materials have decomposability and certain mechanical strength, but due to their lack of bone induction ability and smooth surface, it is difficult for them to provide attachment points for cells. However, HA can induce bone formation, but its mechanical strength is poor and it is easily crushed. Therefore, the 2 are mixed to form a hybrid scaffold that can provide both mechanical strength and bone induction ability. In similar studies, only HA attached to the surface of PCL has functionality. This study used an electron microscope to observe the roughness of the scaffold surface. The higher the HA content, the rougher the surface and the easier it is for cells to adhere and grow, but the mechanical strength decreases accordingly.
BMSCs-M cells are easy to obtain and have the potential to act as osteoblasts, chondrocytes, and adipocytes after induction; therefore, they are considered ideal seed cells [41]. However, there are many hematopoietic stem cells in the bone marrow, leading to difficulties in isolation, identification, and culture. The bone cortex does not contain hematopoietic stem cells. Mesenchymal stem cells isolated from the bone cortex grow well and can avoid contamination by the many hematopoietic stem cells. Flow cytometry showed that CD29 and CD44 were over-expressed and CD34 and CD45 were under-expressed in bone cortical mesenchymal stem cells (BMSCs-C), indicating that the isolated cells were not hematopoietic stem cells and vascular endothelial cells. Osteogenic, adipogenic, and chondrogenic induction showed that mesenchymal stem cells were indeed isolated from the bone cortex. After cells were continuously subcultured to the fifth generation, the morphology of bone marrow mesenchymal stem cells changed significantly and the proliferation rate slowed significantly, while there was less aging of bone cortex mesenchymal stem cells and the proliferation ability was higher than that of bone marrow mesenchymal stem cells. Therefore, BMSCs-C cells were used as seed cells for the seeding of PCL/nHA composite scaffolds in this study.
3D printing technology can be scanned by computed tomography (CT) and magnetic resonance imaging (MRI), and then converted by computer to build a 3D model and then print the required objects with a 3D printer [42]. This study successfully printed the proposed mixed pore-size scaffolds using Solidworks and accurately set the printing conditions using slicing software. Because the high content of nHA is difficult to evenly disperse when mixing PCL, it easily blocks the nozzle and can cause a poor molding effect. In this study, according to the characteristics of the 2 materials after mixing, such as shape appearance, and the results of many pre-experiments, it was found that the shape effect was better when the content of nHA in the mixed composite scaffold was less than 25%. Studies have shown that tissue growth on the porous scaffold prepared by 3D printing is largely determined by the pore size, porosity, and spatial structure of the pores [43]. Therefore, the scaffold used for bone repair must be a 3D structure with good connectivity and an appropriate pore size, to ensure that the biological scaffold has a good cell growth environment, nutrient delivery channel, and angiogenesis conditions [44].
Scanning electron microscopy showed that the 3 scaffolds (300 μm, 500 μm, and 300/500 μm) were full of completely connected pores with sizes from 300 μm to 500 μm, meeting the design requirements. The surface of the scaffold fiber was rough, which is conducive to cell adsorption on the scaffolds. The results also showed that scaffolds with different pore sizes have no significant effect on cell proliferation, which may be because the smaller the pore size, the larger the surface area and the larger the cell attachment space, which can improve the cell proliferation ability [45,46]. However, large pore size is conducive to delivery of nutrients and oxygen, and it also promotes cell proliferation. The number of living cells in each group was significantly higher than that of dead cells after dye staining on day 28, indicating good biocompatibility of the scaffold. Compared to scaffolds, the number of cells on the 1st, 4th, and 7th days was relatively small, which does not reflect the difference in growth space caused by the difference in scaffold porosity and pore size. Cell proliferation ability is mainly related to the difference in cell adhesion performance caused by the microstructure of seed cells and scaffolds. The printing materials of the 3 scaffolds were nHA and PCL mixed in the same proportion, so there was no obvious difference in proliferation ability. However, if the culture is continued, the spatial difference within the scaffold can give more space for cell attachment, changing the ability to promote proliferation.
In terms of mechanical properties, natural bone has strong mechanical properties. The elastic modulus of cancellous bone is about 0.1–0.5 GPa, while that of cortical bone is 12–18 GPa. However, the elastic modulus of composite porous scaffolds in bone tissue engineering is generally 0.12–6.8 MPa, and the yield strength is 0.02–1 MPa [47]. The elastic modulus and yield strength of the 3D printing composite scaffold prepared in the present study basically conform to the elastic modulus range of tissue engineering bone. We also found that the mechanical strength of the material decreases with the increase of the pore size. The 300/500-μm mixed pore size scaffold had the same large pore size of the 500-μm scaffold, but its mechanical properties were slightly lower than those of the 300-μm scaffold.
The early expression of osteoblast differentiation is the secretion of alkaline phosphatase and the formation of calcium. In this study, after 21 days of continuous culture of BMSCs-C cells, the calcium content and alkaline phosphatase activity on 300/500-μm mixed pore size scaffolds were significantly higher than those of the control group and the 500-μm scaffold, and there was no significant difference between these 2 groups. These results suggest that the small pore size is conducive to osteoblast differentiation and alkaline phosphatase secretion [48]. There was a significant difference in the osteogenesis of the scaffolds in these 3 groups, which may also be due to the relatively small surface area of the cells that can be attached due to the large pore size and high porosity and the relatively small number of cells that proliferate after continuous culture, leading to the difference in osteogenesis detection. There was no significant difference between the300/500-μm mixed pore size scaffold and the 300-μm scaffold in the bone formation of the scaffold, which may be due to the short in vitro culture time compared to the in vivo culture, and the effect of promoting material exchange and promoting angiogenesis of the 500-μm pore size scaffold with large aperture was not reflected. If cultured in vivo for 12 weeks, the osteogenesis of the 2 scaffolds may be different. Additionally, the mechanical strength of the scaffold established in this study is still far from that of natural bone tissue. Therefore, these materials can only be used as fillers and can only be used as load-bearing structures when there is sufficient external support.
In the field of orthopedics, tissue engineering is used to fill bone defects and repair cartilage damage. The purpose of this study was to solve the problem of scaffold repair of bone defects. This study only examined the effect of simply changing the pore size composition of the scaffold on osteogenesis. In future research, we will combine bioprinting technology to induce blood vessels to grow through large pores in mixed pore sizes, thereby resolving the problem of insufficient nutrient supply for scaffold seed cells because of the difficulty of blood vessels growing into the scaffold. Furthermore, seed cells can grow fully on the scaffold, resolving the problem of large bone defects.
Although this study provided some interesting results, there were also some limitations. First, because 300/500-μm scaffold has a large pore size, it may have advantages over the 300-μm scaffold in material exchange and vascular growth. This study lacked a comparison between osteogenesis and vascular growth in long-term culture, and animal experiments are needed. Second, gradient printing includes the pore gradient and the material gradient. The ability of nHA alone to induce bone formation is limited, and the mechanical properties of the PCL/nHA combination are low. In this study, only a pore gradient was used, and no material gradient experiment was conducted. In future research, we may consider using a variety of materials to form a material gradient, increase the ability to induce osteogenesis, angiogenesis, and improve the mechanical properties. Third, XRD or FTIR was not used to analyze the addition of HA to the PCL due to the alternative observation methods used in this study.
Conclusions
In this study, 3 polycaprolactone/nanohydroxyapatite (PCL/nHA) composite scaffolds with different pore sizes were constructed using 3D printing technology and bone cortical mesenchymal stem cells (BMSCs-C) as seed cells, all of which had no obvious toxicity. The mechanical properties of the 300/500-μm mixed pore size scaffold with 500-μm pores were slightly lower than those of the 300-μm scaffold, but as a filling material, it needed external support. The 300/500-μm mixed pore size scaffold had the same early osteogenic performance as the 300-μm scaffold and conformed to the interconnected pore structure of natural bone. The large pore size of 500 μm in a 300/500-μm mixed pore size scaffold was conducive to nutrient exchange, which is a better model for building tissue engineering scaffolds. The present study involved scaffold design, printing, cell culture, formation of scaffold cell complexes, and assessment of osteogenic ability, which may provide a theoretical and experimental foundation for subsequent investigations.
Figures
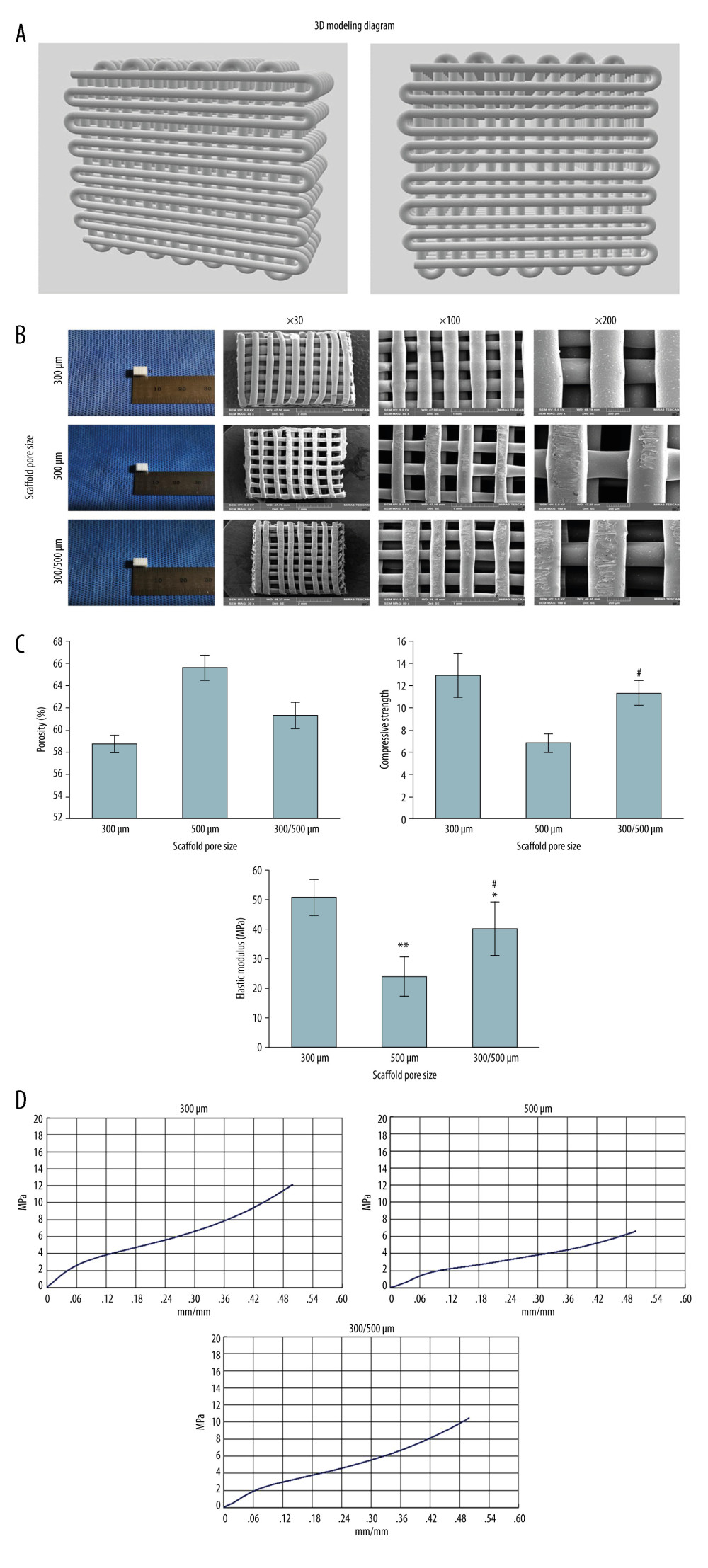
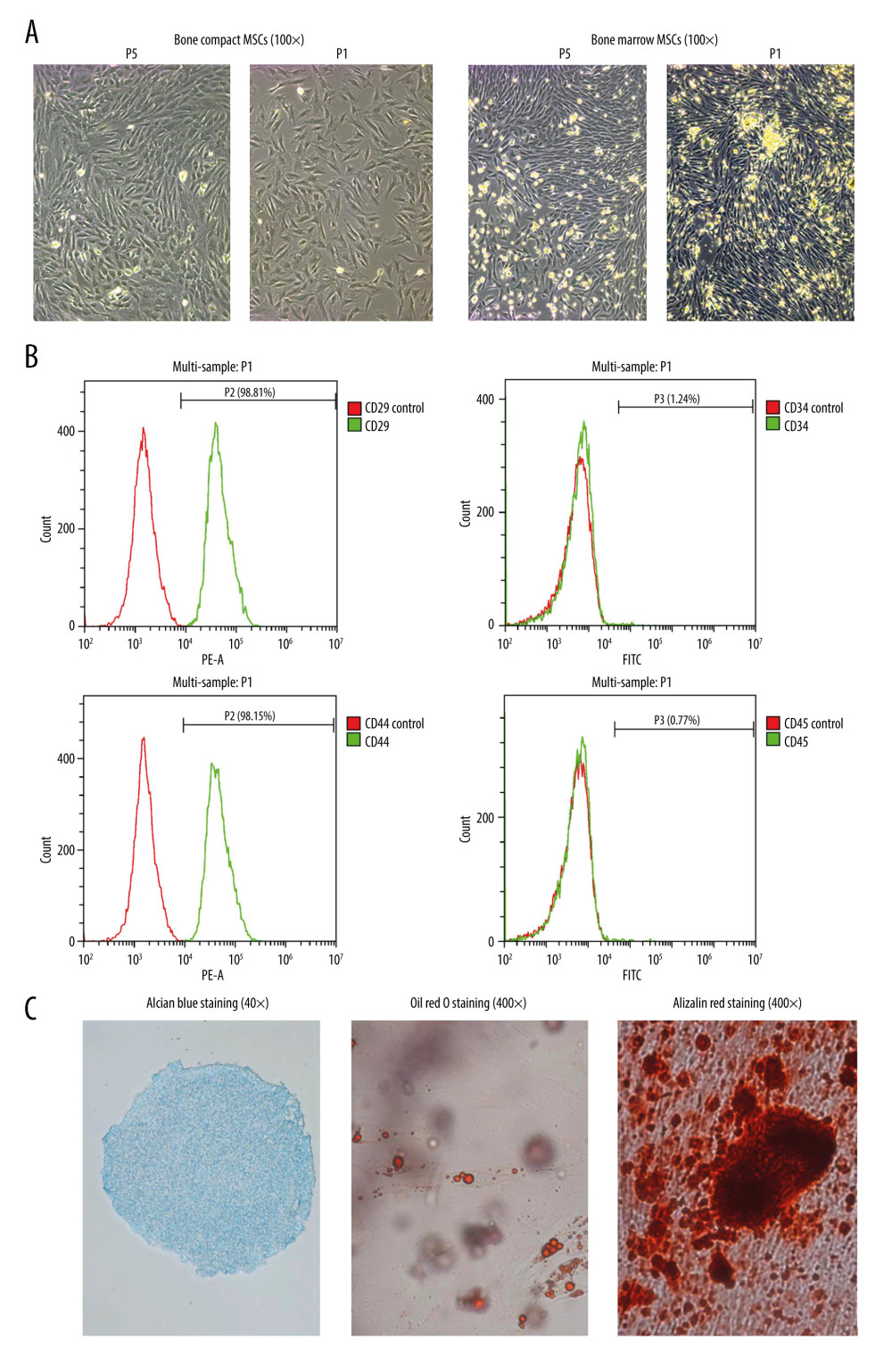
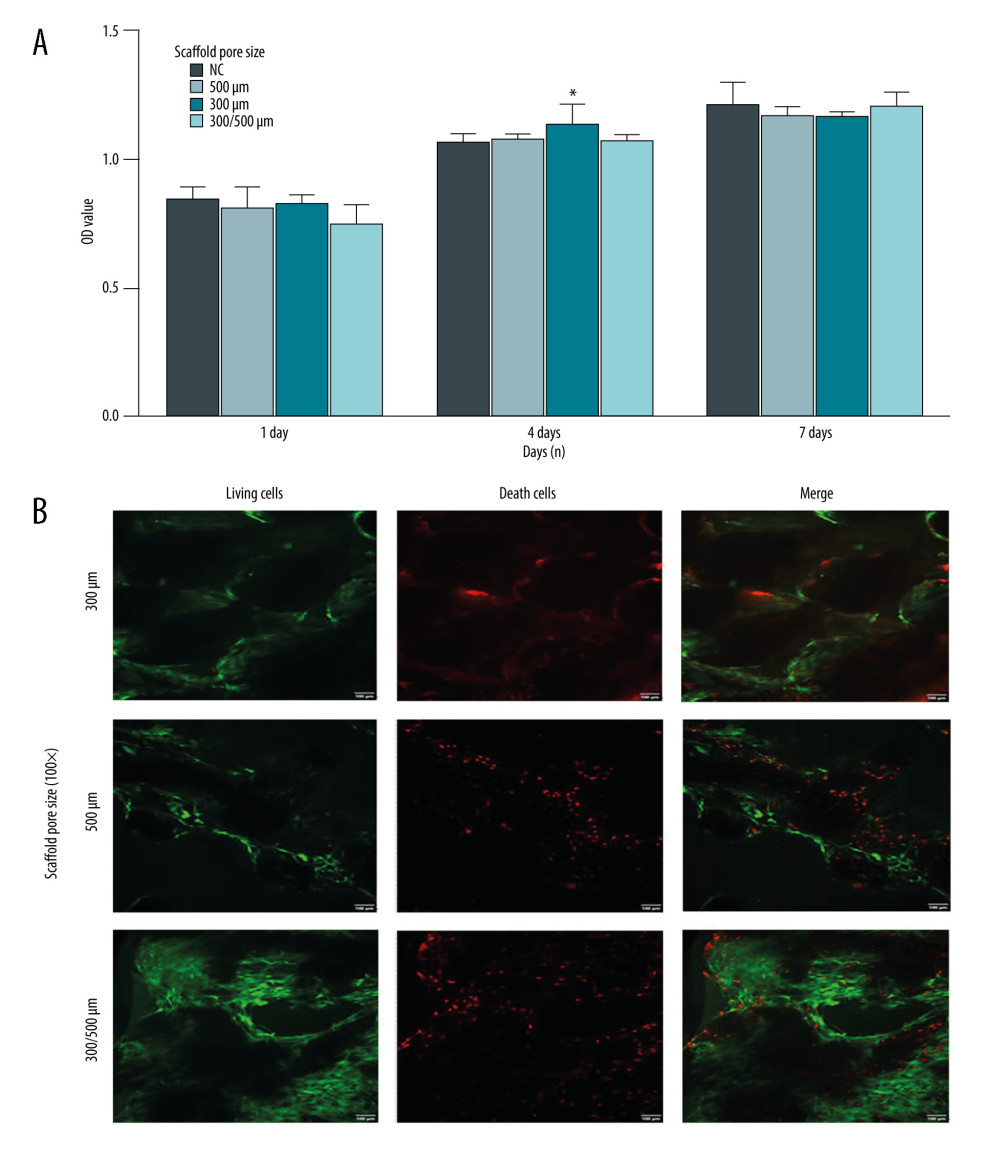
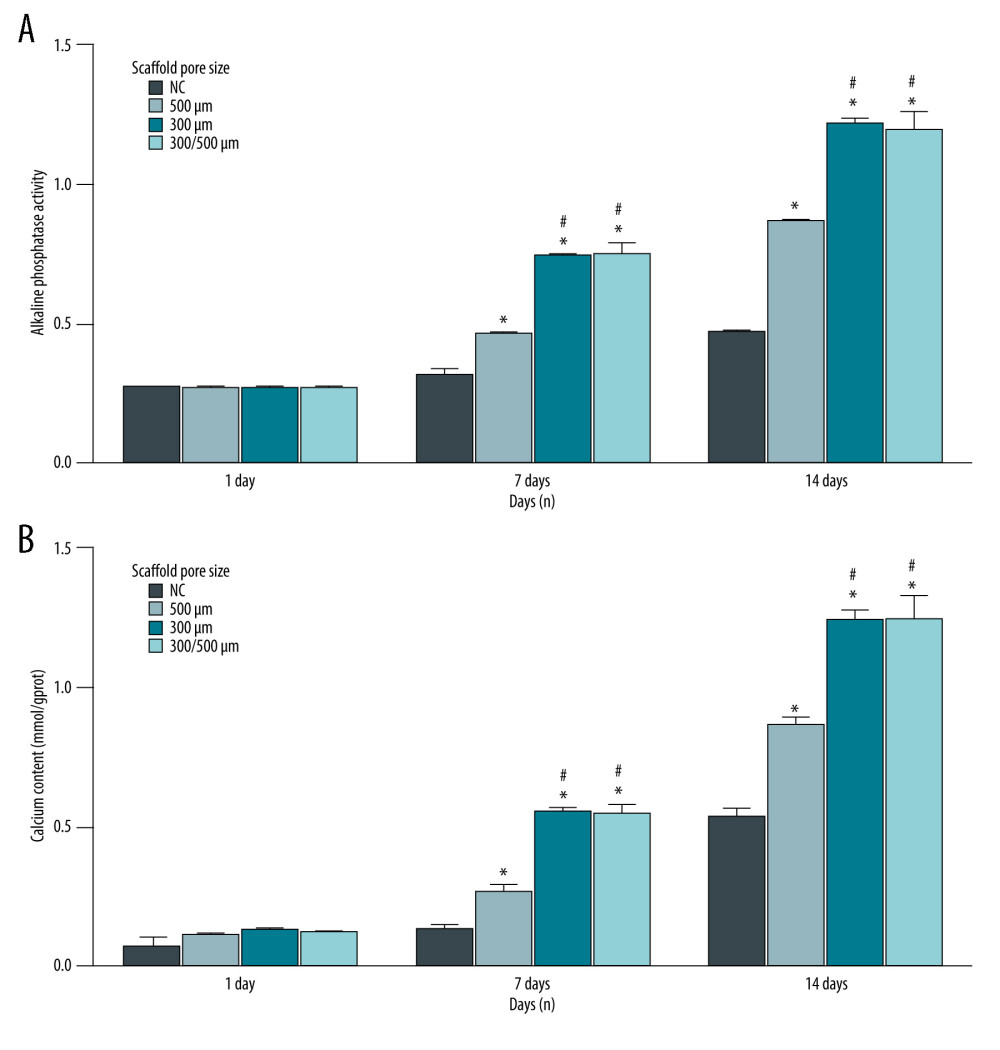
References
1. Zekry KM, Yamamoto N, Hayashi K, Reconstruction of intercalary bone defect after resection of malignant bone tumor: J Orthop Surg (Hong Kong), 2019; 27; 2309499019832970
2. Abay Akar N, Gürel Peközer G, Torun Köse G, Fibrous bone tissue engineering scaffolds prepared by wet spinning of PLGA: Turk J Biol, 2019; 43; 235-45
3. Drăgan E, Nemţoi A, Review of the long-term outcomes of guided bone regeneration and autologous bone block augmentation for vertical dental restoration of dental implants: Med Sci Monit, 2022; 28; e937433
4. Gawroński K, Rzepecki P, Sawicki W, Mobilization of hematopoietic stem cells for hematopoietic cells autologous transplantation with use of plerixafor: Ann Transplant, 2017; 22; 296-302
5. Shi H, Zhou P, Li J, Functional gradient metallic biomaterials: Techniques, current scenery, and future prospects in the biomedical field: Front Bioeng Biotechnol, 2021; 8; 616845
6. Arif ZU, Khalid MY, Sheikh MF, Biopolymeric sustainable materials and their emerging applications: J Environ Chem Engineer, 2022; 10; 108159
7. Xi LC, Li HY, Yin D, Long non-coding RNA-2271 promotes osteogenic differentiation in human bone marrow stem cells: Open Life Sci, 2018; 13; 404-12
8. Sivanathan KN, Gronthos S, Grey ST, Immunodepletion and hypoxia preconditioning of mouse compact bone cells as a novel protocol to isolate highly immunosuppressive mesenchymal stem cells: Stem Cells Dev, 2017; 26; 512-27
9. Wan Z, Zhang P, Liu Y, Four-dimensional bioprinting: Current developments and applications in bone tissue engineering: Acta Biomater, 2020; 101; 26-42
10. Wu X, Jia Y, Sun X, Tissue engineering in female pelvic floor reconstruction: Eng Life Sci, 2020; 20; 275-86
11. Munir MU, Salman S, Javed I, Nano-hydroxyapatite as a delivery system: Overview and advancements: Artif Cells Nanomed Biotechnol, 2021; 49; 717-27
12. Ahmed HY, Safwat N, Shehata R, Synthesis of natural nano-hydroxyapatite from snail shells and its biological activity: Antimicrobial, antibiofilm, and biocompatibility: Membranes (Basel), 2022; 12; 408
13. Khalid MY, Rashid AA, Arif ZU, Recent advances in nanocellulose-based different biomaterials: types, properties, and emerging applications: J Mater Res Technol, 2021; 14; 2601-23
14. Arif ZU, Khalid MY, Noroozi R, Recent advances in 3D-printed polylactide and polycaprolactone-based biomaterials for tissue engineering applications: Int J Biol Macromol, 2022; 218; 930-68
15. Karabulut H, Ulag S, Dalbayrak B, A novel approach for the fabrication of 3D-printed dental membrane scaffolds including antimicrobial pomegranate extract: Pharmaceutics, 2023; 15; 737
16. Chao L, Jiao C, Liang H, Analysis of mechanical properties and permeability of trabecular-like porous scaffold by additive manufacturing: Front Bioeng Biotechnol, 2021; 9; 779854
17. Hayashi K, Shimabukuro M, Kishida R, Structurally optimized honeycomb scaffolds with outstanding ability for vertical bone augmentation: J Adv Res, 2022; 41; 101-12
18. Di Luca A, Ostrowska B, Lorenzo-Moldero I, Gradients in pore size enhance the osteogenic differentiation of human mesenchymal stromal cells in three-dimensional scaffolds: Sci Rep, 2016; 6; 22898
19. Di Luca A, Longoni A, Criscenti G, Toward mimicking the bone structure: Design of novel hierarchical scaffolds with a tailored radial porosity gradient: Biofabrication, 2016; 8; 045007
20. Vijayavenkataraman S, Zhang L, Zhang S, Triply periodic minimal surfaces sheet scaffolds for tissue engineering applications: An optimization approach toward biomimetic scaffold design: ACS Appl Bio Mater, 2018; 1; 259-69
21. Kelly CN, Miller AT, Hollister SJ, Design and structure-function characterization of 3D printed synthetic porous biomaterials for tissue engineering: Adv Healthc Mater, 2018; 7; e1701095
22. Ashammakhi N, Ahadian S, Darabi MA, Minimally Invasive and Regenerative Therapeutics: Adv Mater, 2019; 31; e1804041
23. Vaz VM, Kumar L, 3D printing as a promising tool in personalized medicine: AAPS Pharm Sci Tech, 2021; 22; 49
24. Della Bona A, Cantelli V, Britto VT, 3D printing restorative materials using a stereolithographic technique: A systematic review: Dent Mater, 2021; 37; 336-50
25. Ozyilmaz ED, Turan A, Comoglu T, An overview on the advantages and limitations of 3D printing of microneedles: Pharm Dev Technol, 2021; 26; 923-33
26. Arif ZU, Khalid MY, Noroozi R, Additive manufacturing of sustainable biomaterials for biomedical applications: Asian J Pharm Sci, 2023; 18(3); 100812
27. Khalid MY, Rashid AA, Arif ZU, Natural fiber reinforced composites: Sustainable materials for emerging applications: Results Eng, 2021; 11; 100263
28. Dey M, Ozbolat IT, 3D bioprinting of cells, tissues and organs: Sci Rep, 2020; 10; 14023
29. Ong CS, Nam L, Ong K, 3D and 4D bioprinting of the myocardium: Current approaches, challenges, and future prospects: Biomed Res Int, 2018; 2018; 6497242
30. Yang GH, Yeo M, Koo YW, 4D bioprinting: Technological advances in biofabrication: Macromol Biosci, 2019; 19; e1800441
31. Arif ZU, Khalid MY, Zolfagharian A, 4D bioprinting of smart polymers for biomedical applications: Recent progress, challenges, and future perspectives: React Funct Polym, 2023; 179; 105374
32. Khalid MY, Arif ZU, Ahmed W, 4D printing: technological and manufacturing renaissance: Macromol Mater Eng, 2022; 307; 200003
33. Anandhapadman A, Venkateswaran A, Jayaraman H, Advances in 3D printing of composite scaffolds for the repairment of bone tissue associated defects: Biotechnol Prog, 2022; 38; e3234
34. Khalid MY, Arif ZU, Ahmed W, 4D printing: Technological developments in robotics applications: Sens Actuators A Phys, 2022; 343; 113670
35. Yuan L, Cao J, Hu M, Bone marrow mesenchymal stem cells combined with estrogen synergistically promote endometrial regeneration and reverse EMT via Wnt/β-catenin signaling pathway: Reprod Biol Endocrinol, 2022; 20; 121
36. Chen Z, Wei J, Zhu J, Chm-1 gene-modified bone marrow mesenchymal stem cells maintain the chondrogenic phenotype of tissue-engineered cartilage: Stem Cell Res Ther, 2016; 7; 70
37. Tang W, Zhang H, Liu D, Icariin accelerates cartilage defect repair by promoting chondrogenic differentiation of BMSCs under conditions of oxygen-glucose deprivation: J Cell Mol Med, 2022; 26; 202-15
38. Aonuma T, Tamamura N, Fukunaga T, Delayed tooth movement in Runx2+/− mice associated with mTORC2 in stretch-induced bone formation: Bone Rep, 2020; 12; 100285
39. Su Z, He L, Shang H, Overexpression of bone morphogenetic Protein-1 promotes osteogenesis of bone marrow mesenchymal stem cells in vitro: Med Sci Monit, 2020; 26; e920122
40. Perez RA, Mestres G, Role of pore size and morphology in musculo-skeletal tissue regeneration: Mater Sci Eng C Mater Biol Appl, 2016; 61; 922-39
41. Qu Y, Zhou L, Lv B, Growth differentiation factor-5 induces tenomodulin expression via phosphorylation of p38 and promotes viability of murine mesenchymal stem cells from compact bone: Mol Med Rep, 2018; 17; 3640-46
42. Sultan S, Mathew AP, 3D printed porous cellulose nanocomposite hydrogel scaffolds: J Vis Exp, 2019(146); 59401
43. Gupta D, Vashisth P, Bellare J, Multiscale porosity in a 3D printed gellan-gelatin composite for bone tissue engineering: Biomed Mater, 2021; 16; abf1a7
44. Wang L, Nan X, Hou J, Preparation and biological properties of silk fibroin/nano-hydroxyapatite/hyaluronic acid composite scaffold: Biomed Mater, 2021; 16; ac08aa
45. Chen Z, Yan X, Yin S, Influence of the pore size and porosity of selective laser melted Ti6Al4V ELI porous scaffold on cell proliferation, osteogenesis and bone ingrowth: Mater Sci Eng C Mater Biol Appl, 2020; 106; 110289
46. Han Y, Lian M, Wu Q, Effect of pore size on cell behavior using melt electrowritten scaffolds: Front Bioeng Biotechnol, 2021; 9; 629270
47. Montazerian H, Mohamed MGA, Montazeri MM, Permeability and mechanical properties of gradient porous PDMS scaffolds fabricated by 3D-printed sacrificial templates designed with minimal surfaces: Acta Biomater, 2019; 96; 149-60
48. Jafary F, Hanachi P, Gorjipour K, Osteoblast differentiation on collagen scaffold with immobilized alkaline phosphatase: Int J Organ Transplant Med, 2017; 8; 195-202
Figures
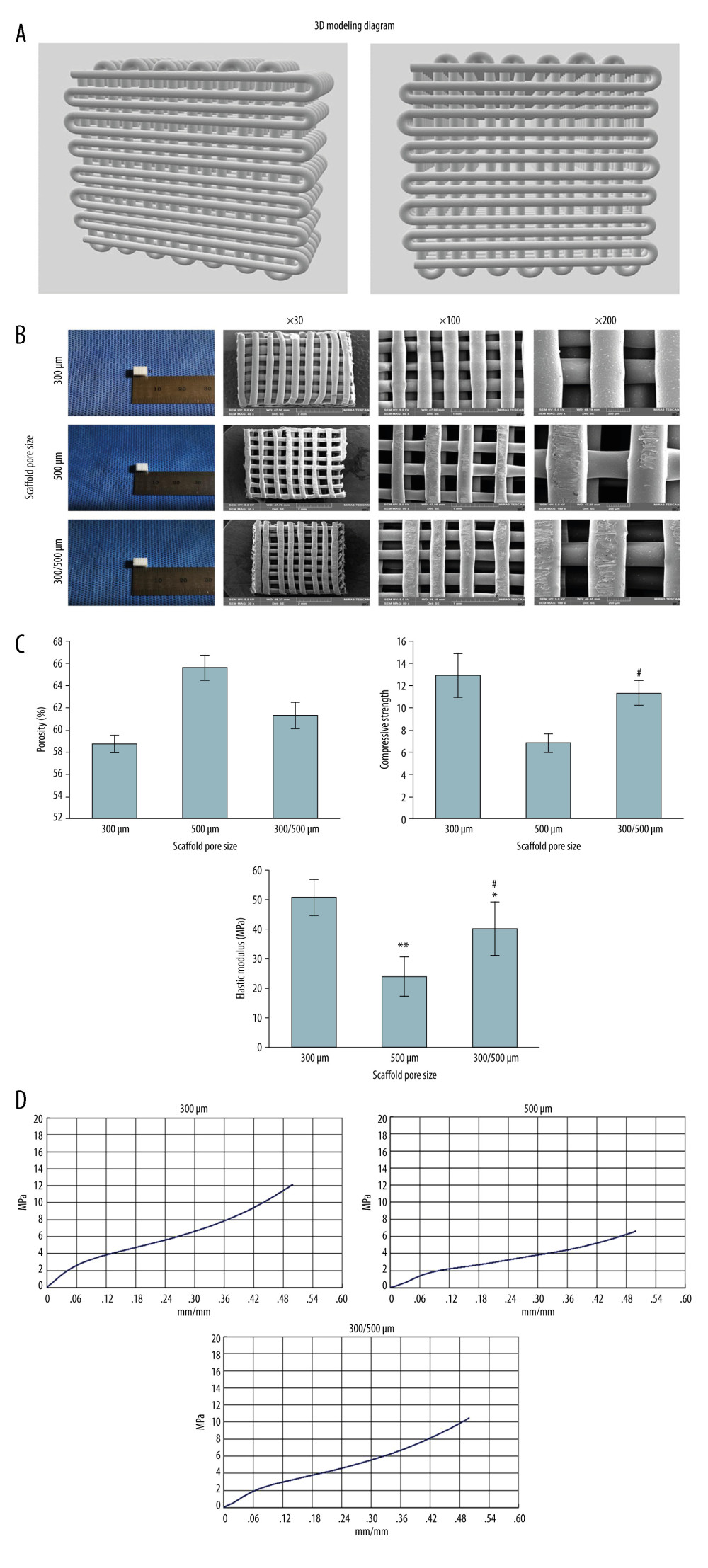
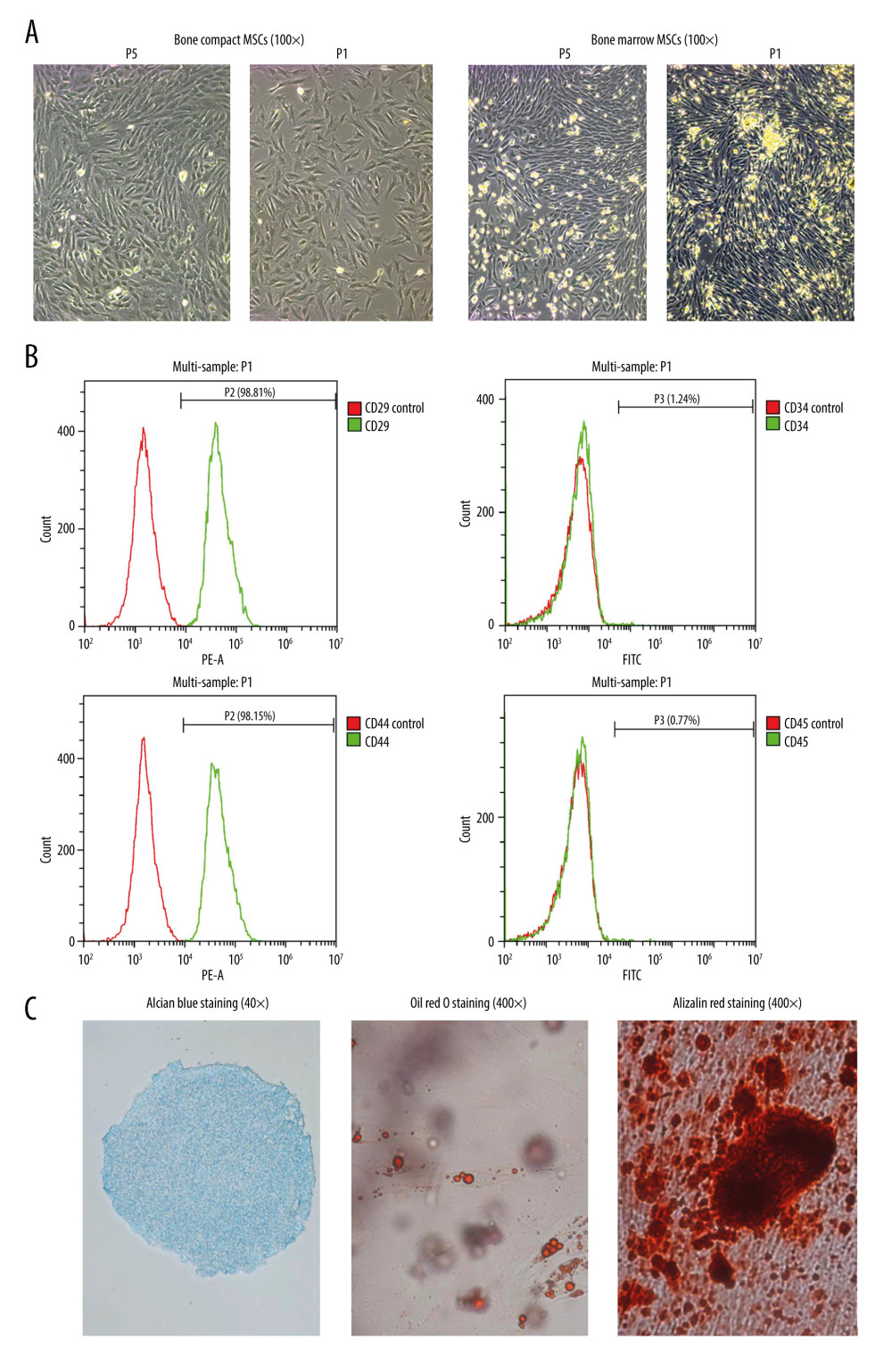
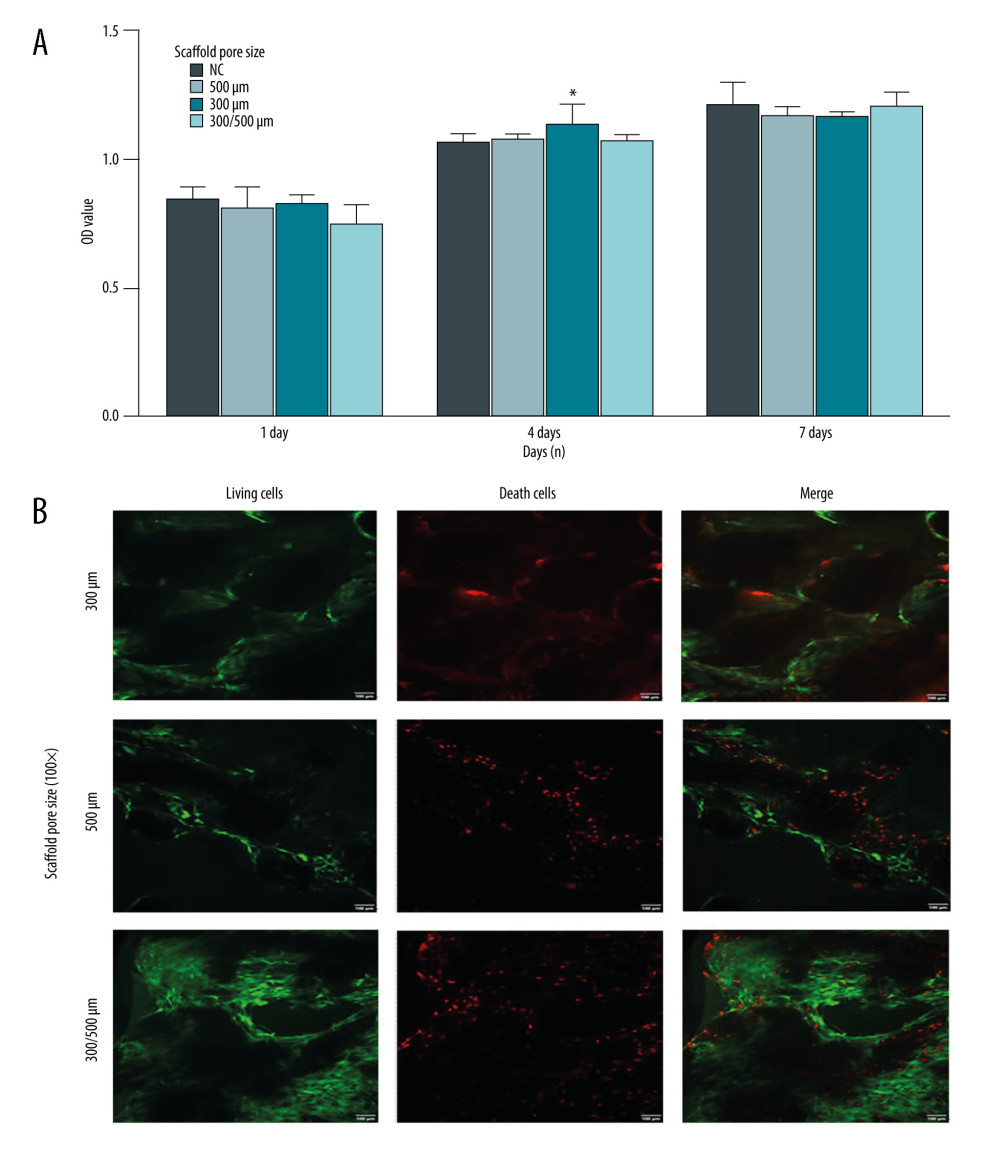
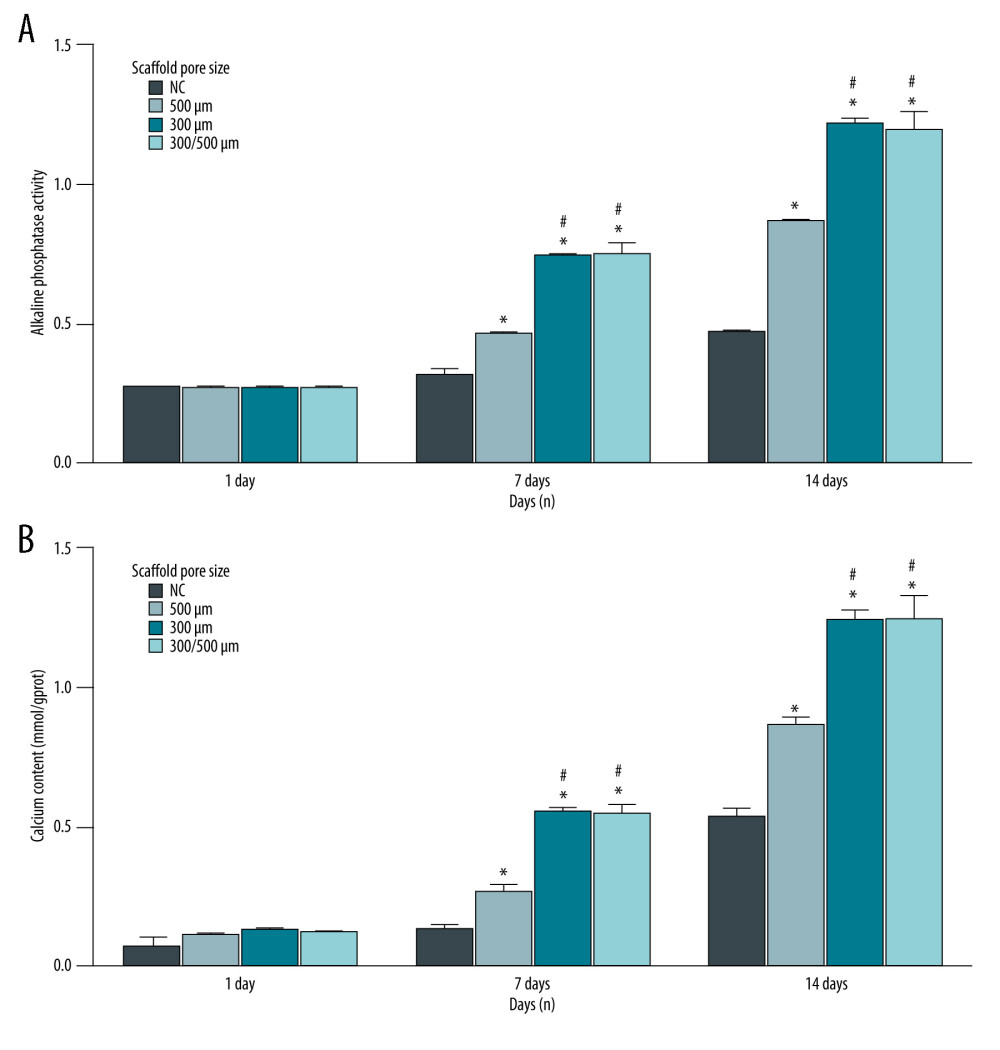
In Press
18 Mar 2024 : Original article
Does Antibiotic Use Increase the Risk of Post-Transplantation Diabetes Mellitus? A Retrospective Study of R...Ann Transplant In Press; DOI: 10.12659/AOT.943282
20 Mar 2024 : Original article
Transplant Nephrectomy: A Comparative Study of Timing and Techniques in a Single InstitutionAnn Transplant In Press; DOI: 10.12659/AOT.942252
28 Mar 2024 : Original article
Association Between FEV₁ Decline Rate and Mortality in Long-Term Follow-Up of a 21-Patient Pilot Clinical T...Ann Transplant In Press; DOI: 10.12659/AOT.942823
02 Apr 2024 : Original article
Liver Transplantation from Brain-Dead Donors with Hepatitis B or C in South Korea: A 2014-2020 Korean Organ...Ann Transplant In Press; DOI: 10.12659/AOT.943588
Most Viewed Current Articles
05 Apr 2022 : Original article
Impact of Statins on Hepatocellular Carcinoma Recurrence After Living-Donor Liver TransplantationDOI :10.12659/AOT.935604
Ann Transplant 2022; 27:e935604
12 Jan 2022 : Original article
Risk Factors for Developing BK Virus-Associated Nephropathy: A Single-Center Retrospective Cohort Study of ...DOI :10.12659/AOT.934738
Ann Transplant 2022; 27:e934738
22 Nov 2022 : Original article
Long-Term Effects of Everolimus-Facilitated Tacrolimus Reduction in Living-Donor Liver Transplant Recipient...DOI :10.12659/AOT.937988
Ann Transplant 2022; 27:e937988
15 Mar 2022 : Case report
Combined Liver, Pancreas-Duodenum, and Kidney Transplantation for Patients with Hepatitis B Cirrhosis, Urem...DOI :10.12659/AOT.935860
Ann Transplant 2022; 27:e935860